By Kent Griffith
July 26, 2022 | Electrifying transportation and bringing electrochemical energy storage onto the grid to support intermittent renewables require colossal magnitudes of raw materials. The world is heading for 3–5 terawatt hours (TWh) of energy storage by 2030. Each TWh will require something like 1.5 million tons of cathode active material and 800,000 tons of anode active material. Will the upstream supply chains of lithium, nickel, cobalt, and graphite be able to meet market demand? Where are the weak points and how can they be solved? Analysts and material suppliers at the International Battery Seminar aimed to address these questions.
Andrew Miller, Benchmark Mineral Intelligence, painted a clear picture of the issues at hand. The number of planned gigafactories has ballooned to more than 200 by 2030 with more new announcements every year. At the same time, the market deficit for lithium by 2030 will be 600,000 tonnes (lithium carbonate equivalent) in the best-case scenario and 1,700,000 tonnes in the worst-case scenario. According to Miller, “[The] lithium deficit by mid-2020s is on track to be as big as [the] total market in 2015.” He also pointed out that it takes up to 25 years to bring a new mine online, whereas material processing and cell manufacturing are more typically 2–4 years. As a result of the mismatch between supply and demand, Simon Moores, CEO of Benchmark, recently noted that lithium is currently 20% of the bill of materials of a lithium-ion battery. 2022 is on track to see the first year-to-year $/kWh increase in the modern lithium-ion battery era.
Lithium sits at ~20% of the cost of a lithium ion battery BOM. New @benchmarkmin data.
Some will remember the days when #lithium was under 5% of the cost.
EV makers will need to get a grip of supply chain to drive this below 10% long term.
Priorities: Supply 1st, price 2nd
— Simon Moores (@sdmoores) June 28, 2022
SQM, a leading lithium producer, is approaching the problem of lithium supply with a two-pronged expansion. First, they plan to increase lithium carbonate production from their brine extraction operation at Salar de Atacama in Chile from 120,000 tonnes/yr in 2021 to 210,000 tons/yr in 2024. They are also targeting an increase in lithium hydroxide from 21,500 tonnes to 90,000 tonnes over the same period. Note that the lithium chloride brine is processed into lithium carbonate and hydroxide not at the salar but near the sea at Antofagasta. Increased lithium hydroxide production will come from doubling the brine-derived product as well as opening a 50,000 tonne/yr spodumene operation in Western Australia. Stefan Debruyne of SQM heavily emphasized the steps their company is taking to minimize the environmental and societal impact of lithium production. According to Debruyne, SQM uses water downstream from the Andean indigenous communities and is targeting large reductions in water usage, brine exaction volume, emissions, and waste. Reducing the amount of brine extracted is counterintuitive given their increased lithium production targets, but Debruyne says it is achievable by extracting a brine that is relatively rich in lithium compared to potassium.
SQM has taken steps to increase transparency by working with the open-source life cycle analysis program GREET (Greenhouse gases Regulated Emissions and Energy in Technologies) and team from Argonne National Laboratory to release an independent analysis of cradle-to-gate emissions and water usage of lithium from Chilean brine and Australian spodumene sources. The results indicate that both lithium carbonate (Li2CO3) and lithium hydroxide (LiOH×H2O) consume far less water and energy and emit less greenhouse gases when produced from brine than from spodumene (Figure 1, Table 1). SQM has also made available an online monitoring tool of Salar de Atacama with hydrogeological and biotic data.
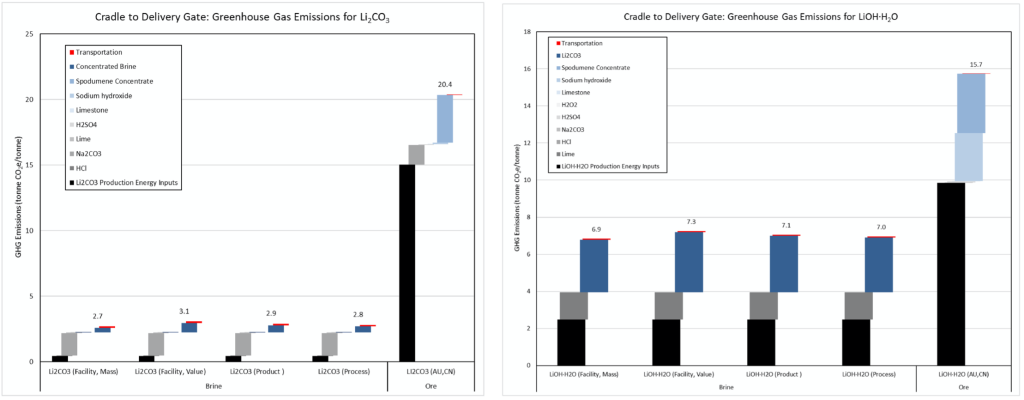
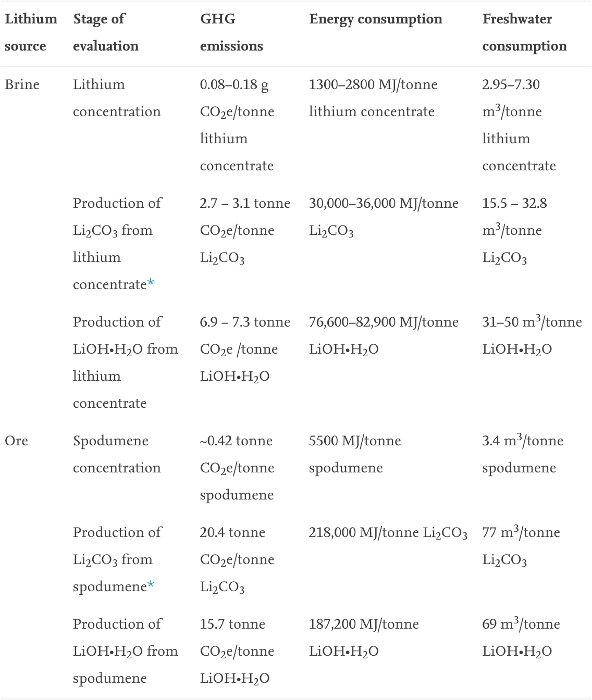
Of course lithium is not the only mineral that will be in high demand as battery cell production scales. Miller noted that nickel, cobalt, and graphite are each going to require $10–50 billion in capital investment to meet battery market requirements. Ken Hoffman, Senior Expert at McKinsey, discussed other factors that will come into play. In his view, the stainless steel industry will reduce its demand for nickel or switch from high-purity Class 1 nickel to low-purity Class 2 nickel, easing pressure on the high-grade supply used by the battery industry. From their polling, range and recharge time are still the top issues for prospective EV consumers. Hoffman does not believe lithium iron phosphate (LFP) will be the major cathode for most markets so focus must be kept on nickel supply. Another avenue on Hoffman’s radar is manganese-based chemistries, which are moving into the spotlight for their lower cost and wider availability than nickel or cobalt, but which will require development of battery-grade manganese plants outside of China.
Speaking of cobalt, Adam McCarthy of the Cobalt Institute, presented on the organization’s efforts to increase transparency and accountability in the cobalt supply chain. The geopolitical, environmental, and humanitarian concerns surrounding cobalt are well-known; 60-70% of the mineral comes from the Democratic Republic of the Congo. According to McCarthy, a majority (70–80%) of this cobalt comes from large-scale mines that are more properly managed, but the remainder is attributed to so-called artisanal miners where safety and child labor are extreme concerns. McCarthy notes that the cobalt market is expected to double from 150,000 tons in 2022 to more than 300,000 tons in 2026, driven almost exclusively by the rechargeable battery market. Recycled cobalt from spent batteries is highly desirable, but the quantities will only be around 34,000 tons by 2030 so further resource development is required and must be done with a focus on sustainability and risk mitigation.
One route to new cobalt and nickel supplies that is being explored is deep-sea mining, as discussed by David Jacoby of Boston University and Duncan Blount of Ocean Minerals. Ocean Minerals is targeting uncovered seafloor nodules that can be harvested directly without overburden removal and with minimal disturbance to the surrounding environment. They have identified a primary cobalt resource near the Cook Islands, which is unique in that most cobalt is produced as a byproduct from copper or nickel mining.
As cell, EV, and ESS production increase, attention must be given to upstream constraints. Batteries are raw material intensive, and mining projects have long timelines. If we want to move away from burning fossil fuels and the associated oil drilling, fracking, and coal mining, we must be willing to increase battery mineral production and aim to do so with minimal environmental impacts and maximal social equity. Recycling will help move the battery industry toward a closed-loop solution, but not in this decade.