By Kyle Proffitt
March 28, 2024 | The International Battery Seminar and Exhibit welcomed more than 2,100 participants to Orlando, Florida, this month, sharing more than 200 presentations covering everything from the latest R&D to discussions of materials sourcing, recycling efforts, and future outlook.
We are always eager to hear Professor Jeffrey Dahn, Dalhousie University, share his latest developments and the outlook for lithium ion batteries. Last year, he reported ultra-long-life batteries made possible in large part by limiting the voltage window for charge and discharge. This year, there was no update on how many additional cycles his batteries had achieved. Instead, he talked about the seemingly benign topic of electrolyte movement. However, in his quest to extend the lifetime of batteries and mitigate concerns that demand will outpace supply, he demonstrated the importance of this seemingly trivial phenomenon.
“Today I want to talk about watching electrolyte move in cylindrical lithium-ion cells and why it matters,” Dahn began. He pointed to a report from 2017 that used neutron diffraction to show electrolyte being squeezed out of the jelly-roll of a cylindrical 18650 cell when fully charged, and he explained that the 10% expansion of graphite during charging is responsible.
Taking an interest in this phenomenon and the potential effects on battery performance and longevity, Dahn and co-workers sent two 18650 cells, one with a graphite anode and one with a SiO-graphite anode, to the Canadian Light Source for high-speed CT imaging. The cells were cycled at a C/3 rate (3 h charge) to 4.2 V and discharged at 1C to 2.5V with time-resolved images taken every 6 minutes.
Movies of this process clearly revealed electrolyte flowing out of the interlayer spaces between electrodes and separators and beginning to fill the core of the cells during charging, with process reversal during discharge. Figure 1 shows a representation of this electrolyte movement.
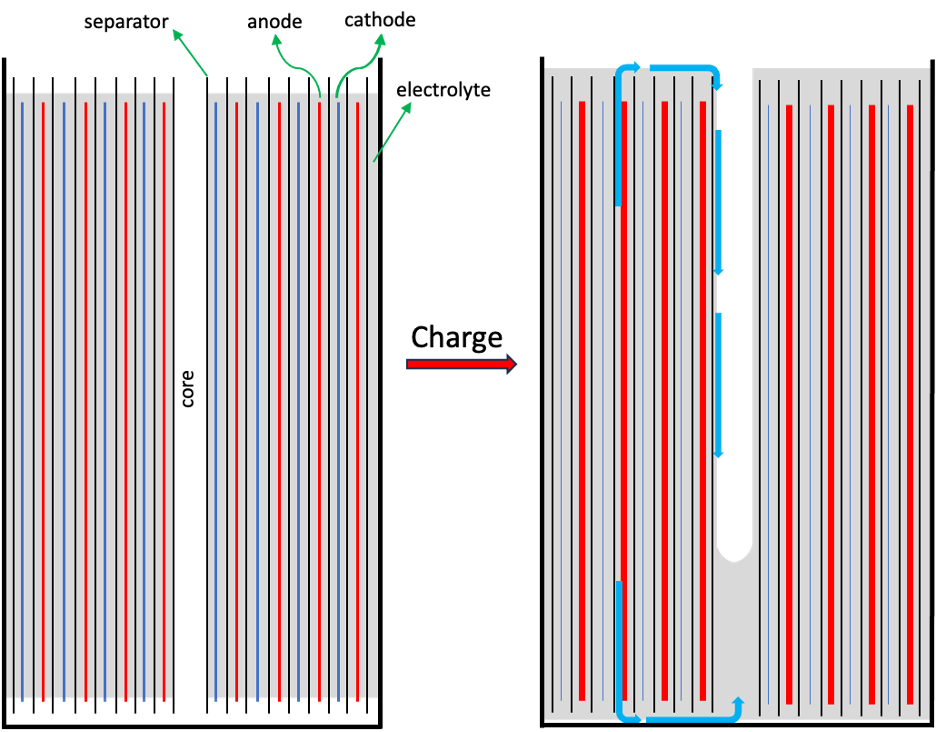
With graphite anodes, the electrolyte filled approximately 1/8 of the core, but this increased to 3/8 with SiO, as expected from the greater SiO expansion during lithium intercalation. Dahn asked the audience, “is it important or is it just an interesting movie?”
He thinks it is important, because ultimately this electrolyte movement creates gradients that limit battery performance, especially with high-volume-change anode materials like silicon and rapid charge/discharge cycles, and there are practical implications. For one, cells in a horizontal configuration undergo greater capacity fade.
Dahn demonstrated with 2170 cells and blamed electrolyte pumping for the changes. Importantly, he also showed that reducing the voltage window (a move he recommended in last year’s talk) provides the batteries with similar longevity independent of orientation. Dahn did not elaborate on why electrolyte pumping is worse for horizontal batteries, but he pointed out that electrolyte circulation can occur in the vertical setup. In contrast, it is possible that electrolyte can become trapped within the core when operated horizontally and not circulate as easily.
Based on this result, Dahn gave some advice: “don’t mix the orientations of cylindrical cells if they have large volume change materials… they’ll fade at different rates.”
Where Does the Gradient Come From?
Explaining gradient formation, Dahn alluded to a talk earlier in the day by Johannes Wandt of BMW, in which he also discussed salt inhomogeneity and a reduction in electrolyte concentration from 1.2 to 0.6 molar at the ends of a battery after just 18 fast-charge cycles.
Wandt explained in his presentation how a cathode-to-anode gradient of the electrolyte salt LiPF6 is established during charge, and this works in concert with the mechanical anode expansion and electrolyte squeezing to disrupt homogeneity. The electrochemical process of charging pulls the salt toward the cathode, meaning that the LiPF6 concentration is lower near the anode precisely when it is being pushed out of the ends of the jelly roll from this compartment. During discharge the situation reverses such that the salt concentration becomes higher at the anode. The mechanical effect of electrolyte being sucked back into the top and bottom of the jelly roll as the anode contracts will then pull more salt-depleted electrolyte into the ends. The net effect is that the center of the jelly roll becomes enriched while the ends become more depleted.
“This is an additive effect, and things get worse and worse as cycling proceeds,” Dahn said. It’s further exacerbated because the salt concentration can equilibrate rapidly between the 150-micron anode-cathode distance. However, Dahn says, it takes weeks to equilibrate over the 65 mm height of the jelly roll.
And again, that matters. “The concentration at the ends can become quite small, and then you end up being susceptible to lithium plating at the edges of the jelly roll,” Dahn said.
Can’t Afford a Synchrotron?
Dahn’s group wanted to study this electrolyte movement without accessing multi-million-dollar equipment, so they developed a simpler apparatus to reveal internal material movement. Their design relies on the concept of moment of inertia (I), an object’s resistance to rotation around an axis. Imagine placing a cylindrical battery horizontally on a table and spinning it. When the mass is uniformly distributed, the moment of inertia is relatively low at the ends of the battery, but if sufficient electrolyte pools at the top and bottom of a cell, more force will be required to rotate it, and I increases. Based on this principle, Dahn’s group built a torsional oscillator and measured the resonant frequency f—where the oscillator vibrates maximally, which is inversely related to the square root of I. Much of this work was published in 2023.
Using electromagnets to induce alternating motion over a range of specific frequencies, they could identify the frequency at which the battery rotates maximally—which occurs within only about 1 degree, requiring decently sensitive angle sensors. They then monitor how this resonant frequency changes as batteries undergo cycles of charging and discharging, revealing the same electrolyte pumping phenomenon.
Their apparatus was surprisingly sensitive in detecting resonant frequency changes; Dahn walked the audience through a potential explanation. In the uncharged, relaxed state, f is highest and I is lowest. During charge, f decreases and I increases as electrolyte moves to the ends of the battery. Applying a constant voltage hold at the top of the charge state, though, f increases back to an intermediate level. This is thought to reflect electrolyte filling the inner core, moving back toward the midpoint of the battery, and thus reducing I. Then with a rapid discharge, f rapidly decreases, which must reflect electrolyte being sucked back into the ends of the jelly roll and having higher electrolyte density at the ends. The rapid decrease in f may also support the idea that some electrolyte remains trapped in the core nearer the ends. The faster the discharge, the further f decreases. A constant low voltage hold after discharge then required approximately 40 minutes before f returned to its initial, highest value. This reflects the time necessary for electrolyte to diffuse throughout the jelly roll structure and is an indicator that skipping this hold could be detrimental.
Dahn says this simple machinery will be useful in determining how best to minimize the negative effects that electrolyte pumping could have on battery performance. His group has also designed equipment for studying 4680 cells, which are more difficult to monitor with X-ray CT due to their size, and for testing cells in a vertical orientation.
Dahn closed with more practical advice. “The faster that you charge, the worse the impacts are.” He also implied that, based on his findings, it would be prudent to choose a shorter cell if large volume-change materials are involved.