By Kent Griffith
October 7, 2022 | An overarching goal in lithium-ion battery research and development is to increase cathode and anode energy density without severely sacrificing cycle life, shelf life, charge rate, or safety. Semiconductors and solar cells operate on the basis of electron transfer. Batteries, on the other hand, operate by storing charge through the physical transfer of ions (e.g., lithium) in addition to electrons between electrodes. Thus, it is difficult to break free from the inherent trade-off in battery operation of increased volumetric change as a function of increased energy density.
Compare two leading lithium battery anode candidates as an example: graphite stores around 370 mAh/g and expands by just over 10% in the reaction from C6 to LiC6, whereas silicon stores 3600 mAh/g and expands by about 300% going from Si to Li3.75Si. It is possible to use less than the maximum capacity of silicon to keep the volume change in check, a strategy that is widely pursued by silicon anode companies. The same situation is true for cathodes, especially common nickel-rich cathode materials like NCA and NMC811 (Figure 1), which see typical volume changes of 6-7%. Large volume changes are associated with particle cracking and film delamination that increase cell resistance, decrease usable energy, and eventually lead to cell failure. In nickel-rich cathodes, specifically, the volume changes can be enough to break contact between the smaller primary particles that agglomerate to create larger secondary particles (Figure 2).
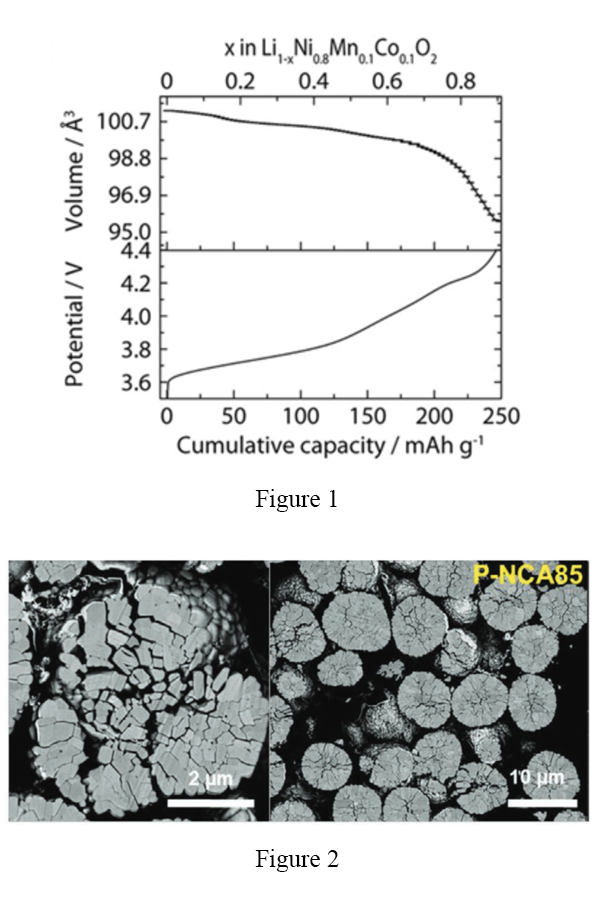
A new paper out in Nature (DOI: 10.1038/s41586-022-05115-z) reports an approach to break the trend between volume change and energy density with cobalt-free high-entropy oxides. In this work, Rui Zhang, Chunyang Wang, Peichao Zou, and Huolin Xin from the University of California, Irvine and co-workers from four different national laboratories collaborated to explore the layered oxide structure that is common to LiCoO2, NMC, and NCA, but without cobalt and instead with the concurrent addition of many dopants. Their cathode composition, LiNi0.8Mn0.13Ti0.02Mg0.02Nb0.01Mo0.02O2, relies on some of the most common dopants used for cathode stabilization. Surprisingly, the combination of these dopants leads to a structure that changes volume by only 0.3% when cycled to 4.3 V (about 200 mAh/g). The authors thus refer to this material as “zero strain”, a term used elsewhere in the community for electrodes with less than 1% volume change, such as the lithium titanium oxide (LTO) anode. The high-entropy cathodes also exhibit good rate performance, including fast charging of 170 mAh/g in 12 minutes (Figure 3) and safety characteristics that are competitive with safer, lower-nickel-content cathodes.
Regarding the role of the dopants, the authors found that Ti, Nb, and Mo segregate at least partially to the grain boundaries, whereas Mg is uniformly distributed throughout the material. This suggests that Ti, Nb, and Mo may induce effects from coating (surface stabilization) as well as doping (bulk stabilization), in line with work from Nobel Laureate Stan Whittingham and others. Xin and co-workers suggest that the high-entropy doping strategy is more generalizable and works with other nickel compositions (e.g., 60–90% Ni) and other dopants (e.g., Al, Zr, Cr). Although these other systems typically showed volume changes of a few percent and thus would not be considered zero-strain, they outperformed undoped analogues with regard to volume change.
Shashwat Anand, a battery researcher at the University of California, Berkeley, who was not involved in this work, carefully evaluated the new publication and noted that, “It seems like the non-Ni/Mn/Co cations are playing multiple roles here. In different cathodes like the disordered rocksalt phases, small volume changes are expected (Joule, DOI: 10.1016/j.joule.2022.05.018). The mechanism here appears to be different—perhaps the dopants are able to absorb local changes as lithium is inserted and extracted without the need for a bulk structure change. This is surprising and the mechanism requires further study.” He also noted that high-entropy oxides have been previously studied for the stabilization of sodium-ion battery cathodes with the same layered structure as in the work here (Angewandte Chemie International Edition, DOI: 10.1002/anie.201912171).
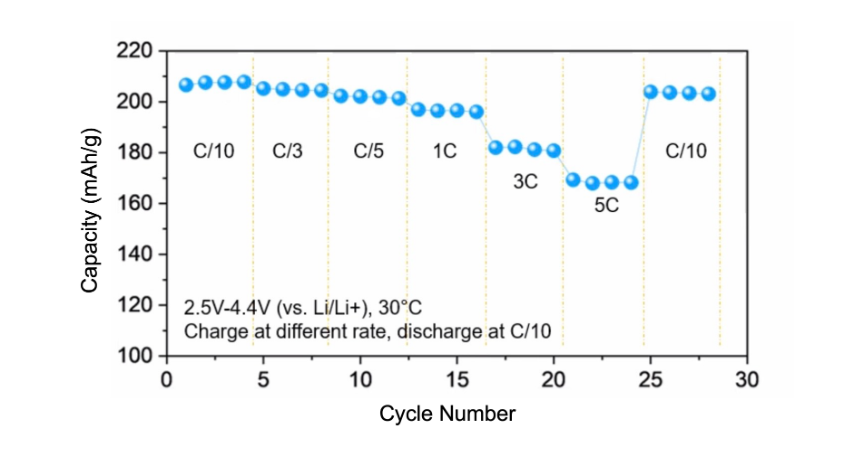
What does the future hold for these high-entropy lithium-ion battery cathodes? In the paper, Xin reported two US non-provisional patent applications and a PCT application filed by the University of California, Irvine, on the basis of that work. He is in the process of forming a startup and raising a pre-Series A round now. In an email, Xin said, “We are working on commercialization of the zero-Co zero-strain cathode. We have two products in the pipeline: one with high-Ni content aimed at replacing NMC-811 for power-intense EV applications and one with low-Ni content aimed at displacing NMC-532 for less-power-demanding applications. Our materials have extremely fast charge rate, ultralong cycle life, and ultrahigh thermal stability—these properties making them the ultimate choice for future EV batteries—particularly for those more conservative EV makers.”
Ulderico Ulissi, Battery Research Lead at Rho Motion, who was not involved in the work, commented that, “The manuscript is an example of good practice in development of novel positive electrode active materials. It shows convincing proof-of-concept measurements, elucidating some of the chemo-mechanical properties of high entropy, low strain, Co-free materials. Development of longer cycle life, safer active materials should be considered a priority, and the strategy is a move in the right direction. The work should be considered as seminal, showing a promising strategy rather than a series of breakthrough materials.”
As with any new battery technology, there is work to be done. Ulissi added, “Performance, safety, and power characteristics will need to be demonstrated in larger, graphite-based prototypes with lean electrolyte (e.g., in multilayer cell stacks) and benchmarked against state-of-the-art material (e.g., single crystal NMC) for mass-produced positive electrode active materials, not prepared in a controlled laboratory environment. It will be interesting to see if this specific approach could enable, for example, low (or zero) nickel, zero cobalt materials—this could possibly be initially explored via in-silico approaches.”